Laser Induced Electron Diffraction: An emerging technique for unravelling molecular dynamics
Written by: Prabhash Prasannan Geetha
December 15, 2017
Investigating the structure of molecules has a fundamental role in basic science. In order to fathom the dynamics during a chemical reaction we must study the motion of nuclei and electrons in atoms and molecules on their fundamental time scales. To do this we need to develop new tools that are capable of probing a molecule at a spatial resolution of an angstrom and a temporal resolution of a few tens of femtosecond.[1 – 4] In the past few decades, X-rays and electron diffraction techniques have been widely used to study molecules with sub-angstrom spatial resolution. However these techniques are, in general, not capable of probing the molecular dynamics down to femtosecond (~ 10-15 second) or attosecond (~ 10-18 second) time scales, the timescales at which reactions may occur. Indeed, the temporal resolution of conventional electron diffraction is limited to hundreds of femtoseconds because of the Coulomb repulsion in the electron bunch. This hundreds of femtoseconds temporal resolution is not sufficient to conduct a detailed study of the chemical dynamics and initiation reactions. [5, 6]
Laser Induced Electron Diffraction (LIED) is a very promising technique for achieving both spatial and temporal imaging of molecules at appropriate resolutions. This technique is based on probing the structure of a molecule using its own electrons. When a molecule is interacting with a femtosecond laser field, whose magnitude is comparable to the internuclear coulombic fields, a temporally confined electron wave packet can be released from the molecule, near the peak of the electric field, due to tunnel ionization. In this way an electron wave packet is accelerated into the continuum by the external electric field.

Figure 1. Schematic representation of laser induced electron diffraction principle. Black dashed lines correspond to the low momentum events recorded from the direct electrons. Magenta dashed lines correspond to the high momentum events caused by re-scattered electrons. (Adopted from Ref. 4 with permission)
A portion of this electron wave packet drifts away from the core of the molecule and goes directly to the detector. These electrons will appear as ‘low momentum events’ with maximum energy of 2Up (black dashed line in the Figure 1), where Up is the ponderomotive energy of the free electron in the laser field. A fraction of the electron wave packet is driven back and scatters through the parent ion. This elastically scattered electron carries structural information about the molecule and produces diffraction patterns due to the interference between amplitudes arising from different scattering centres constituted by the nuclei. The maximum energy of these re-scattering electrons can go up to 10Up (magenta dashed line in Figure 1).[7] From the electron momentum angular distribution, we can extract information about the nuclei using quantitative re-scattering theory (QRS) [3 - 6]. This approach can be extended for the study of molecular dynamics.[6] For time-resolved experiments using LIED, the temporal resolution is given by the convolution between pump and probe pulse while the spatial resolution is related to the de Broglie wavelength of the electron wave packet. If the de Broglie wavelength of the returning electron wave packet becomes comparable with the molecular dimensions, we can extract inter atomic spacing.[3,4] Thus, to extract structural information with the highest resolution, we need to accelerate electrons into very high energies. Since Up is proportional to the intensity and to the square of the wavelength of the driving pulse, we can increase the electron energy by increasing these quantities. We are dealing with fragile molecules having low ionization potentials and thus if we increase the intensity of the driving pulse too much it will destroy the molecule. We can however achieve a good spatial resolution in by increasing the wavelength of the driving pulse. For this reason we use longer driving wavelengths in LIED experiments.
This LIED technique may provide the opportunity for observing changes in nuclear structure and highly occupied molecular (HOMO) orbitals concomitantly. There are several studies of LIED using Cold Target Recoil Ion Momentum Spectroscopy (COLTRIMS), and the results show that this technique is powerful for studying the dynamics of complex molecular systems.[4, 6, 8] Specifically, COLTRIMS allows the reconstruction of the 3D momentum distribution of photoelectrons. In our laboratory, we want to further adapt this approach by acquiring the photoelectrons with a ‘Velocity Map Imaging’ (VMI) spectrometer which detects the 2D projection of the electron momentum distribution. VMI offers reduced technical complexity and significantly higher collection efficiency per pulse.
The research of our group in the Institute for Photonics and Nanotechnologies of CNR (CNR-IFN), mainly focuses on ultrafast molecular imaging using High-order Harmonic Generation (HHG) and photoelectron spectroscopy (Figure 2). LIED is complementary with respect to HHG spectroscopy techniques.
My research under the supervision of Dr. Caterina Vozzi and Prof. Salvatore Stagira, focuses on the development of molecular imaging based on LIED. This requires the development of techniques for manipulating both the alignment and orientation of molecules since random molecular alignment in the investigated sample would hinder the retrieval of the molecular properties.[3] The aim of the project is realization of static and time-resolved measurements of the diffracted electrons in relatively complex molecules, such as hydrocarbons, by VMI. As a first step we will investigate LIED static imaging of simple molecules, such as O2, N2, N2O, CO, OCS. These experiments will be used as a benchmark for the experimental approach. The same technique will then be used to undertake static imaging of more complex molecules. Then we will develop a pump-probe setup for the realisation of time resolved LIED.
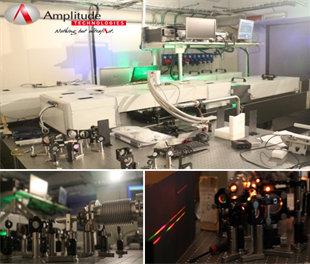
Figure 2. Udyni lab @ CNR IFN
A UV-OPA will be developed for pumping the molecules, for instance for triggering dissociation dynamics in the target. A mid-IR pulse from a tunable high energy Optical Parametric Amplifier (OPA) at 1 kHz will be used for the photoionisation. The lab UDynI is equipped with a Ti:Sapphire driving laser at 800 nm with time duration of 22 fs and 15 mJ energy at 1 kHz repetition rate (Amplitude Technologies). The home-made Carrier Envelop Phase (CEP) stabilized OPA is tunable in the range of 1.2 to 2 μm with pulse duration of <20 fs with 2-mJ energy at 1 kHz repetition rate. To perform LIED, we also developed a ‘home-made’ velocity map imaging (VMI) spectrometer for electron momentum detection that can detect the photoelectrons with energy up to 200 eV (Figure 3).
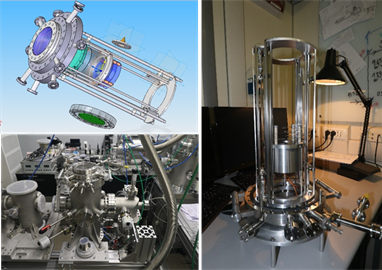
Figure 3. The VMI spectrometer installed in CNR-IFN UDynI lab. This home-made VMI is capable of detecting photoelectrons of energy up to 200 eV.
Our results will contribute to the development of molecular movies (direct visualization of orbital dynamics during structural changes) with both sub-angstrom spatial resolution and sub-femtosecond temporal resolutions.
Personal experiences as an ASPIRE Early Stage Researcher (ASPIRE ESR)
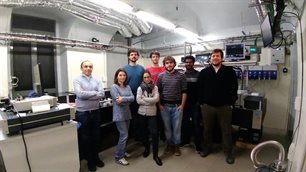
Figure 4. Researchers @ lab UDynI in CNR-IFN Milan.
As an ASPIRE ESR I have the opportunity to access a network of leading scientists and to access cutting-edge laboratories working in photoelectron spectroscopy. Furthermore, as an ASPIRE ESR I can undertake secondments at external institutes/companies to improve my skillset. I also network regularly with other highly motivated ASPIRE ESRs working in similar fields. Every day I face novel, interesting challenges in the laboratory and my colleagues consistently encourage me to further push the boundaries of my research. I strongly believe that future training available to me from the ASPIRE programme – both academic and industrial - will allow me to thrive as a research scientist.
Figures
Figure 1: schematic representation of laser induced electron diffraction principle. Black dashed lines correspond to the low momentum events recorded from the direct electrons. Magenta dashed lines correspond to the high momentum events caused by re-scattered electrons. (adopted from Ref. 4 with permission)
Figure 2: Udyni lab @ CNR IFN
Figure 3: The VMI spectrometer installed in CNR-IFN UDynI lab. This home-made VMI is capable of detecting photoelectrons of energy up to 200 eV.
Figure 4: Researchers @ lab UDynI in CNR-IFN Milan.
References
- T. Zuo et al., Chemical Physics Letters 259, 313 (1996).
- A Staudte et al., APS Division of Atomic, Molecular and Optical Physics Meeting Abstracts, (2008).
- M. Meckel et al., Science 320.5882 (2008), 1478.
- C.I. Blaga et al., Nature 483.7388 (2012), 194.
- Z. Chen et al., Phys. Rev. A 79, 033409 (2009).
- M.G. Pullen et al.,Nature Communications 6, 7262 (2015).
- M. Peters et al.,Phys. Rev. A 83, 051403(R) (2011).
- Y. Ito et al., Struct. Dyn. 3, 034303 (2016).